Johnson Offers Keen Insight on Turbulent Flows in Physics Today
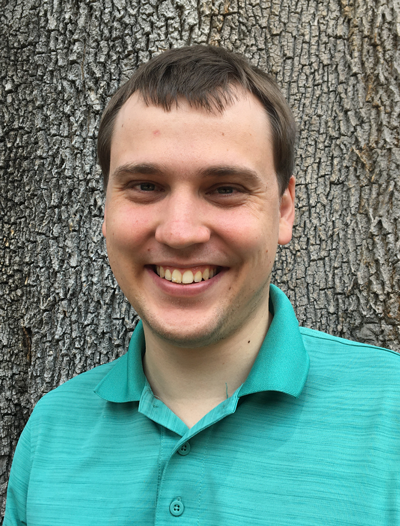
May 11, 2021 - Turbulence isn’t just what compels us to white-knuckle clench and brace ourselves as airplane passengers. A fluctuating flow of fluid (gas or liquid), turbulence is all around us. Beyond creating panic in the skies, it affects everyday weather patterns, the production of energy we use, the air we breathe, how we play and watch sports, space travel, and even the blood that courses through our hearts.
Perry Johnson, UC Irvine assistant professor of mechanical and aerospace engineering, explores turbulent flows in “The Squeezes, Stretches and Whirls of Turbulence,” published in the April 2021 issue of Physics Today.
“Turbulent flows are characterized by apparently random, chaotic motions,” wrote Johnson in the article. “And they are everywhere.”
Understanding the physics of turbulent flows has applications in fields like aerodynamics, renewable energy, atmospheric science, oceanography and healthcare. They affect gas turbine engines, large-scale wind farms, deep-sea oil spills, coughs and sneezes (pertinent to the spread of COVID-19), and more.
However, understanding and modeling fluid turbulence proves difficult because of its nonlinear features that span a wide range of sizes. This can result in physically impossible simulations or extremely expensive computational costs of brute-force simulations due to large amounts of data. Therefore, the development of simplifying models is vital for engineers and scientists studying turbulent flows.
“Turbulent flows generate coherent velocity fluctuations – ‘squeezes, stretches and whirls’ as I call them,” said Johnson. “I use the example of a large-scale wind farm, which can be many kilometers in length but – if you zoom in – the flow is filled with tiny millimeter-size features and all sizes in between. One of the most fundamental questions among those studying turbulence is: How do all these motions with such different sizes get energized?”
Two processes are at the core of the question. In his research, Johnson traced the classic process known as the “energy cascade.” He highlighted “vortex stretching,” which occurs when a tornado-like motion stretches along its axis. Johnson likened it to a figure skater pulling in their arms while spinning, conserving angular momentum, which causes a stretched vortex to spin faster. This generates more energized motions at smaller scales, happening randomly all over and causing a chaotic turbulent flow. What results is an overall cascade of energy from larger motions to smaller ones.
In the last few decades, some researchers have posed an alternative energy cascade mechanism called strain self-amplification to explain how energy passes from larger to smaller motions. This occurs when a strong compressive strain rate steepens as faster-moving pieces of fluid overtake slower-moving ones in their paths. “The effect is analogous to an ocean wave steepening before it breaks,” he explained.
Johnson’s work tackles the question of how important either of the two processes are to the energetics of turbulence. “Previous attempts to quantify this relied on looking at only the largest term in an infinite sum of terms,” said Johnson. “This left some doubt as to the role of the neglected terms.
“My unique contribution was to specify an exact way to distinguish the roles of these two mechanisms, including the role of multiscale interactions. Ultimately, this revealed a somewhat nuanced picture in which both processes play a role, even if one is somewhat stronger than the other.”
Under-resolved computer simulations of turbulent flows are very common in many fields – aerospace, propulsion, energy, oceanography and atmospheric sciences (including studies of Mars), explained Johnson. “In the example of the wind farm, a full resolution of the flow over kilometers with one grid point per millimeter would require something like 1018 grid points (1 with 18 zeros after it)! That's not practical. So it's really useful to develop physically accurate techniques for simulating at partial resolution.”
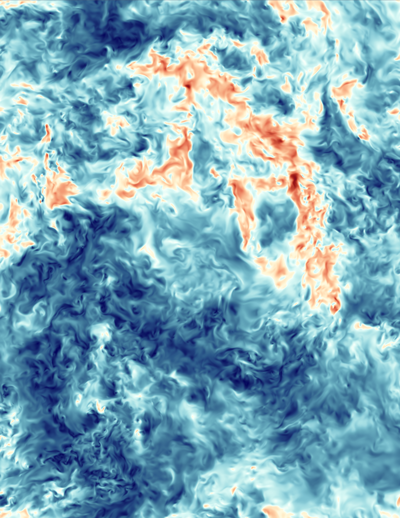
Johnson employed the Navier-Stokes equation, which “encapsulates the law of momentum conservation for a fluid flow,” and spatial filtering to simplify the simulation process. Similar to lowering the resolution of an image, spatial filtering applies a low-pass spatial filter. This blurs the small-scale motions to more clearly highlight the larger ones. In essence, spatial filtering results in the ability to quantify energy transfer rates amongst various scales of motion.
“When you simulate a turbulent flow with insufficient resolution, it is important to have an accurate model taking care of how energy is moving around, particularly how it would be moving to motions smaller than the grid size,” said Johnson.
“A more complete understanding of the physics involved in the energy cascade gives more guidance in constructing these models. A new perspective on how to approach under-resolved turbulence simulations is emerging from my work – viewing this under-resolution as the outcome of a nature-inspired process rather than simply an image-processing trick. This change in perspective facilitated the fundamental insight I outlined above – and I'm excited to see what this idea can do for simulation methods in the coming years here at UCI.”
– Tonya Becerra